Miniature Celestial Bodies
ยฉ NASA/ESA/STScI/G. Bacon
In 1906, Danish astronomer Ejnar Hertzsprung introduced a term that marked a pivotal moment in stellar classification. Observing the reddest stars, categorized as K and M in the Harvard scheme, Hertzsprung discerned a notable dichotomy. Some of these stars appeared significantly brighter than the Sun, while others appeared notably fainter. To differentiate between these two distinct groups, he coined the terms “giant” for the brighter stars and “dwarf” for the fainter ones, thus laying the foundation for modern stellar classification.
Dwarf stars are celestial bodies of average or low luminosity, mass, and size. They are characterized by their position on the Hertzsprung-Russell (H-R) diagram, which plots the temperature of a star against its luminosity. Dwarf stars are located in the H-R diagram, where most stars are found. Dwarf stars encompass a category known as main-sequence stars, which includes our own Sun. These stellar dwarfs exhibit a spectrum of colours spanning from blue to red, indicative of their surface temperatures that range from high (above 10,000 K) to low (a few thousand K). They are classified into several subclasses based on their temperature and luminosity, including white dwarfs, red dwarfs, and brown dwarfs.
CHARACTERISTICS AND PROPERTIES

- Size and Mass: Dwarf stars are defined by their relatively small size and mass compared to other types of stars. They typically range from a fraction of the mass of the Sun to about 0.8 solar masses. Despite their smaller size, they can still exhibit a wide range of physical characteristics and behaviours depending on their mass and evolutionary stage.
- Temperature and Colour: The temperature and colour of dwarf stars are closely related and vary depending on their spectral type and evolutionary status. Dwarf stars can span a broad range of colours, from blue to red. Blue dwarfs have higher surface temperatures, typically above 10,000 Kelvin, while red dwarfs have lower surface temperatures, often ranging from a few thousand Kelvin. This colour variation indicates the stars’ surface temperatures, with blue stars being hotter and red stars being cooler.
- Luminosity: Dwarf stars emit varying levels of light, known as luminosity, which is determined by factors such as surface temperature and size. Red dwarf stars, despite their relatively low surface temperatures, can have low to moderate luminosity due to their smaller size and lower energy output. White dwarf stars, on the other hand, can be surprisingly luminous, particularly in their earlier stages when they are still radiating residual thermal energy from their previous fusion processes.
- Longevity: The lifespan of a dwarf star depends largely on its mass and spectral type. Red dwarf stars, which are low-mass and relatively cool, have the potential to burn steadily for trillions of years. They are considered some of the longest-lived stars in the universe. White dwarf stars, after exhausting their nuclear fuel, can remain luminous for billions of years before gradually cooling and fading into stellar remnants.
- Planetary Systems: Dwarf stars, particularly red dwarfs, are often associated with hosting planetary systems. Their long lifespans and stable energy output make them potential hosts for habitable exoplanets. However, their proximity to their host stars and potential tidal locking may present challenges for planetary habitability.
TYPES OF DWARF STAR

Dwarf stars encompass a variety of stellar types, each with its own unique characteristics and properties. The types of dwarf stars include:
RED DWARF
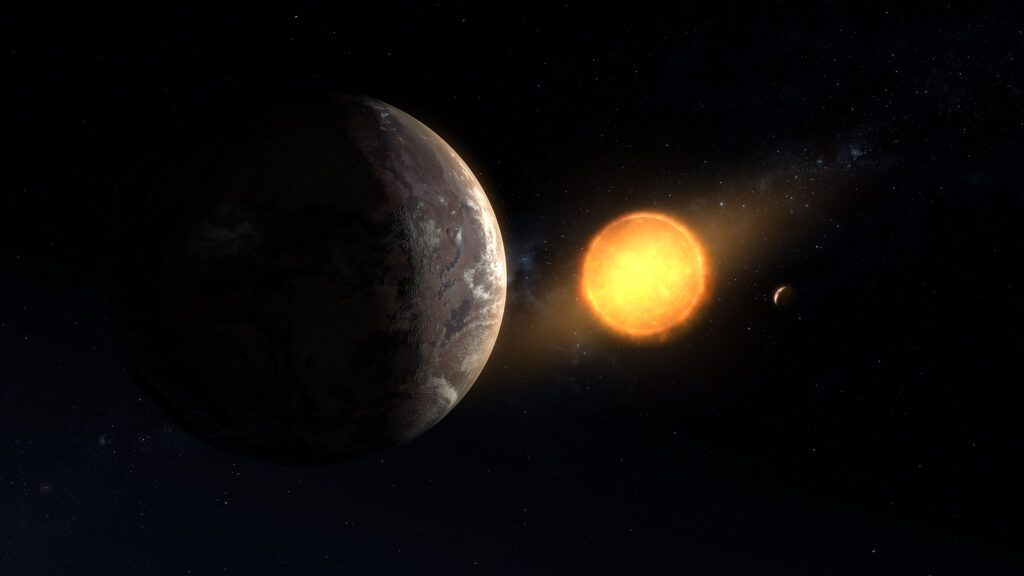
Red dwarfs, the universe’s most prevalent star type, are diminutive hydrogen-burning giants. With masses ranging from 0.08 to 0.6 times that of the Sun, they boast surface temperatures of 2,000-3,500 K, marking them as the coolest main-sequence stars. Their spectral signatures reveal molecules like titanium oxide, a telltale sign of their cooler nature. Despite their diminutive size, they shine with the faintest luminosities, measuring merely 0.0001 to 0.1 times that of the Sun. Red dwarfs endure for trillions of years, marked as spectral type M on the Hertzsprung-Russell diagram, where they inhabit the coolest end of the main sequence.
Red dwarfs are also the smallest type of stars, with radii of about 0.1-0.4 times that of the Sun. They have low surface gravities and low pressures, which result in low fusion rates and low temperatures. The energy generated by red dwarfs is primarily from the fusion of hydrogen into helium in their cores, but the process is slowed down and even prolonged compared to larger stars. Red dwarfs have a region around their cores where convection does not occur, which allows them to burn their entire supply of hydrogen. This results in a long lifetime, with some red dwarfs expected to last for trillions of years.
Red dwarfs are common in binary or larger star systems, and they are the most common type of star in the Milky Way galaxy, making up about three-fourths of the stars in the galaxy. They are also the most common type of star in elliptical galaxies. The nearest star to the Sun is a red dwarf called Proxima Centauri, which is about 4.24 light-years away.
Red dwarfs are important in the search for habitable exoplanets because their low luminosities and small sizes make it easier to detect planets in orbit around them. The habitable zone, or “Goldilocks zone,” around a red dwarf star is much closer to the star than it is around a larger star like the Sun. This means that planets in the habitable zone of a red dwarf star would have shorter orbital periods and would be more likely to transit the star, making them easier to detect with current technology.
WHITE DWARF

White dwarfs mark the final phase in the lifecycle of medium-mass stars, boasting a mass akin to the Sun but a volume akin to Earth. These entities exhibit extraordinary density, approximately a million times denser than water, upheld by the degeneracy pressure of their electron gas interiorsโa resistance generated by electron compression during stellar contraction. The equilibrium structure of white dwarfs, elucidated through Fermi-Dirac statistics and special relativity, unveils a distinctive mass-radius correlation, indicating smaller radii for higher masses. The Chandrasekhar limit defines the threshold mass, around 1.4 solar masses, beyond which stable white dwarf formation is untenable, a crucial prediction in stellar evolution.
White dwarfs are composed of one of the densest forms of matter known, surpassed only by other compact stars such as neutron stars, quark stars, and black holes. They are supported only by electron degeneracy pressure, causing them to be extremely dense. The physics of degeneracy yields a maximum mass for a non-rotating white dwarf, the Chandrasekhar limit, which is approximately 1.44 times the mass of the Sun.
White dwarfs are formed when a red giant star sheds its outer layers and forms a planetary nebula, leaving behind a dense, hot, and luminous core surrounded by a glowing spherical shell. This core is the remnant white dwarf. Usually, white dwarfs are composed of carbon and oxygen ( CO white dwarf). If the mass of the progenitor is between 7 and 9 solar masses, the core temperature will be sufficient to fuse carbon but not neon, in which case an oxygen-neon-magnesium ( ONeMg or ONe) white dwarf may form. Stars of very low mass will be unable to fuse helium; hence, a helium white dwarf may form by mass loss in binary systems.
The material in a white dwarf no longer undergoes fusion reactions, so the star has no source of energy. As a result, it cannot support itself by the heat generated by fusion against gravitational collapse but is supported only by electron degeneracy pressure, causing it to be extremely dense. The average density of matter in a white dwarf must therefore be, very roughly, 1,000,000 times greater than the average density of the Sun, or approximately 10^6 g/cm^3, or 1 tonne per cubic centimetre. A typical white dwarf has a density of between 10^4 and 10^7 g/cm^3.
White dwarfs, discovered for their extreme density, reveal insights into their mass through binary systems like Sirius B or 40 Eridani B. By 1910, Sirius B’s mass was estimated at 0.94 solar masses, aligning closely with modern approximations of 1.00 solar masses. Their high temperatures, deduced from spectral analysis, indicate relatively low absolute luminosity, hinting at their exceptional density. Calculating surface area and radius from known luminosity and distance yields a startling realization: Sirius B and 40 Eridani B are remarkably dense, a revelation that initially puzzled astronomers, showcasing the intricacies of stellar physics and observational techniques.
BROWN DWARF

Brown dwarfs are a class of celestial objects that are intermediate in mass between stars and planets. They are characterized by their inability to sustain hydrogen fusion, which is the primary energy source for stars. Brown dwarfs have masses ranging from about 13 to 80 times the mass of Jupiter, which is the largest planet in our solar system. They are also known as “failed stars” because they do not have enough mass to ignite hydrogen fusion and become full-fledged stars.
Brown dwarfs are classified by their spectral type, which is a measure of their temperature and surface composition. The spectral types for brown dwarfs are M, L, T, and Y, with M being the hottest and Y being the coolest. The spectral type is determined by the presence and strength of certain absorption lines in the object’s spectrum, which are indicative of specific elements and molecules.
Brown dwarfs, distinguished by their subdued luminosity, lack the energy output of stars due to the absence of hydrogen fusion. Instead, they rely on deuterium fusion, converting deuterium (a heavy hydrogen isotope) into helium. However, this process is only viable in brown dwarfs exceeding 13 Jupiter masses. These dwarfs exhibit notably low surface temperatures, ranging from a few thousand degrees Celsius for the hottest specimens to just a few hundred degrees for the coolestโsignificantly cooler than the Sun‘s 5,500-degree Celsius surface. This dual characteristic of low luminosity and temperature underscores the distinct nature of brown dwarfs in the stellar hierarchy.
Brown dwarfs, despite their modest mass, share Jupiter’s size due to electron degeneracy pressure, preventing gravitational collapse. This quantum effect, arising from electron occupation limitations, sustains them. Although lacking hydrogen fusion’s energy output, they persist. Brown dwarfs also exhibit variability, with many displaying brightness fluctuations attributed to expansive surface spots akin to solar sunspots. Though less common than stars, they’re abundant, especially in young star-forming regions and binary systems. Their genesis often involves the fragmentation of collapsing gas and dust clouds, leading to their presence in various celestial configurations.
Despite their many similarities to stars, brown dwarfs are not considered to be full-fledged stars because they do not undergo hydrogen fusion. Instead, they are classified as sub-stellar objects, along with planets and other celestial bodies that are intermediate in mass between stars and planets.
SUBDWARF

Subdwarfs are a class of star that is fainter than a star of the same spectral type on the main sequence, but which is brighter than a white dwarf. They are defined as stars with luminosity 1.5 to 2 magnitudes lower than that of main-sequence stars of the same spectral type. Subdwarfs appear to lie below the main sequence on a Hertzsprung-Russell diagram, which plots the temperature of a star against its luminosity.
There are two main categories of subdwarfs: hot (blue) subdwarfs and cool (red/pink) subdwarfs.
HOT SUBDWARFS
Hot subdwarfs, of bluish spectral types O and B, are an entirely different class of object than cool subdwarfs and are also called “extreme horizontal-branch stars”. They represent a late stage in the evolution of some stars, caused when a red giant star loses its outer hydrogen layers before the core begins to fuse helium. Single subdwarfs may be the result of a merger of two white dwarfs or gravitational influence from substellar companions. B-type subdwarfs, being more luminous than white dwarfs, are a significant component in the hot star population of old stellar systems, such as globular clusters and elliptical galaxies.
Heavy metal subdwarfs are a type of hot subdwarf star with high concentrations of heavy metals, including germanium, strontium, yttrium, zirconium, and lead. Known heavy metal subdwarfs include HE 2359-2844, LS IV-14 116, and HE 1256-2738. The early universe had a low content of elements heavier than helium and formed stars and brown dwarfs with lower metallicity. Only later supernovae, planetary nebulae, and neutron star mergers enriched the universe with heavier elements.
COOL SUBDWARFS
Cool subdwarfs (of spectral types G to M) produce their energy from hydrogen fusion, but their underluminosity is due to their low metallicity, which decreases the opacity of their outer layers and decreases the radiation pressure, resulting in a smaller, hotter star for a given mass. The lower opacity also allows them to emit a higher percentage of ultraviolet light for the same spectral type relative to a main-sequence star of the same spectral type, a feature known as the ultraviolet excess.
Cool subdwarfs are usually members of the Milky Way‘s halo and have high space velocities relative to the Sun. Cool subdwarfs of spectral type L and T also exist, for example, ULAS J131610.28+075553.0 with spectral type sdT6.5. Subclasses of cool subdwarfs include cool subdwarfs, extreme subdwarfs, and ultra subdwarfs.
YELLOW DWARF

Yellow dwarf stars, also known as G-type main-sequence stars, are medium-sized stars that are between 0.84 and 1.15 times the mass of our Sun. The Sun is an example of a yellow dwarf, which has a mass of 1.9891 x 10^30 kg and a diameter of 1,392,000 kilometers. These stars are yellow or white in colour and have surface temperatures ranging from 5,200 to 6,000 degrees Kelvin. The “yellow” bit of the name is a bit misleading, as the colour of these stars can be anything from white to only a little bit yellow.
Yellow dwarf stars are characterized by their hydrogen fusion, where hydrogen atoms combine to form helium, releasing energy in the process. This energy is what makes the star shine. The Sun, as a yellow dwarf, has a core temperature of about 15 million Kelvin (about 15 million Celsius) and a core diameter of about 400,000 kilometers. The core is where the hydrogen fusion takes place, and it is consuming fuel (and losing mass) at the rate of 4 million tonnes per second.
The life span of a yellow dwarf is said to be ten billion years, and it glows brighter as years go by. Its hydrogen fuses into helium, which makes its core hotter. Its energy eventually surmounts its gravity while it grows bigger and turns into a red giant. Ultimately, the yellow dwarf will turn into a white dwarf, a star that has collapsed and turned cooler.
Learn about Sunโs lifecycle here.
Yellow dwarf stars are the most hospitable to life next to K-type stars, given their length of the main sequence, levels of ultraviolet radiation in their habitable zone, the semi-major axis of the inner boundary of this region, and the distance to their tidal locking limit, among other factors. The Earth, orbiting a yellow dwarf, represents the only known example of planetary habitability. The main goal in the field of exoplanetology is to find an Earth analogue planet that meets its main characteristics, such as size, average temperature, and location around a star similar to the Sun.
ORANGE DWARF

Orange dwarf stars, also known as K-type main-sequence stars, are a type of star that may be candidates for supporting extraterrestrial life. They are known as “Goldilocks stars” because they emit enough radiation in the non-UV ray spectrum to provide a temperature that allows liquid water to exist on the surface of a planet, and they remain stable in the main sequence longer than the Sun by burning their hydrogen slower, allowing more time for life to form on a planet around a K-type main-sequence star.
These stars have a habitable zone, ranging from 0.1-0.4 to 0.3-1.3 astronomical units (AU) from the star, depending on the size of the star. This is often far enough from the star so as not to be tidally locked to the star, and to have a sufficiently low solar flare activity not to be lethal to life. In comparison, red dwarf stars have too much solar activity and quickly tidally lock the planets in their habitable zones, making them less suitable for life.
The odds of complex life arising may be better on planets around K-type main-sequence stars than around Sun-like stars, given the suitable temperature and extra time available for it to evolve. Some planets around K-type main-sequence stars are potential candidates for extraterrestrial life.
Orange dwarf stars, slightly dimmer than the Sun, offer advantageous conditions for exoplanet detection as planets crossing their faces become more discernible. With a slightly smaller mass than the Sun, these stars exhibit greater gravitational perturbation when planets orbit them, facilitating detection through observable wobbles. Notably long-lived compared to the Sun, orange dwarfs extend potentially habitable periods for their planets. While the Sun nears its midpoint of a 10-billion-year lifespan, its expansion threatens Earth‘s habitability within 1 to 2 billion years. In contrast, orange dwarfs remain stable for 15 to 45 billion years, offering extended windows for the emergence of complex life.
BLUE DWARF

Blue dwarf stars are a theoretical class of stars that are predicted to develop from red dwarf stars after they have exhausted much of their hydrogen fuel supply. Red dwarfs are fully convective, which means that their entire hydrogen supply can be fused, rather than just that in the core. As a result, red dwarfs are predicted to have lifespans of trillions of years, which is much longer than the current age of the universe.
When a red dwarf star has exhausted most of its hydrogen fuel, it is predicted to increase its radiative rate by increasing its surface temperature, rather than expanding like larger stars do. This increase in surface temperature causes a large shift in the star’s light towards the bluer side of the electromagnetic spectrum, even though the star may not necessarily become blue. The temperature of a blue dwarf star would be comparable to the temperature of the Sun.
Blue dwarf stars are not expected to exist at present because the universe is not old enough for any red dwarfs to have reached this stage yet. They are a theoretical concept based on models of stellar evolution. It is believed that once a blue dwarf star has exhausted its hydrogen fuel, it will become a white dwarf, which is a stellar remnant that is no longer a star. White dwarfs eventually cool and lose their remnant heat, becoming black dwarfs, which is also a theoretical concept because the universe is not old enough for any stellar remnants to have cooled to “black”.